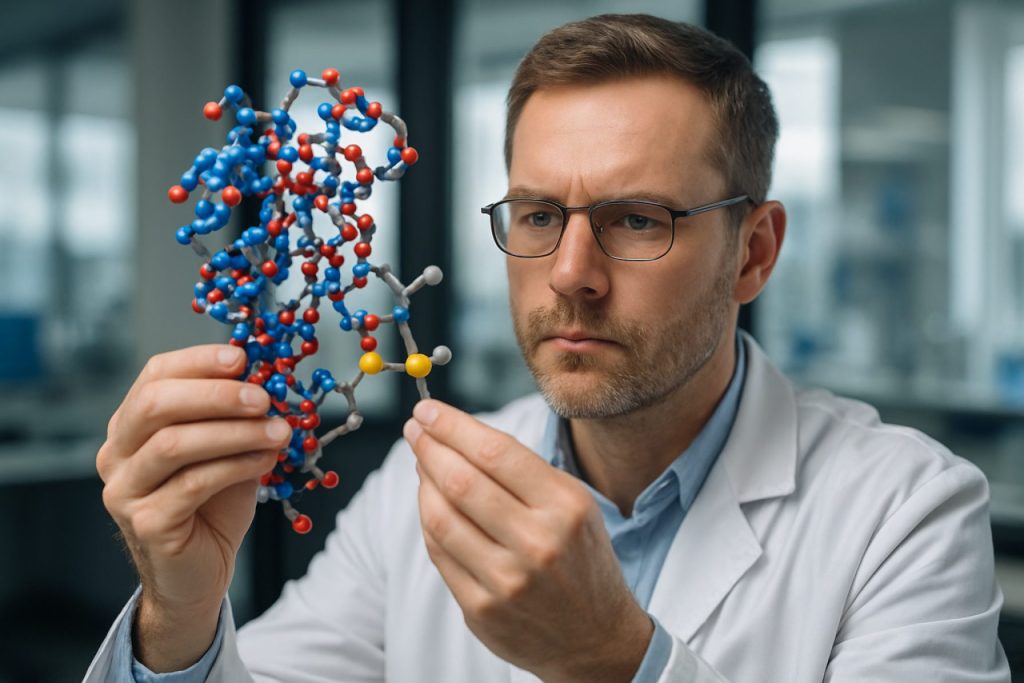
Disulfide Bond Engineering in Protein Design: Transforming Stability, Function, and Therapeutic Potential. Discover How Precision Bonding is Shaping the Future of Protein Science. (2025)
- Introduction: The Role of Disulfide Bonds in Protein Structure
- Historical Milestones in Disulfide Bond Engineering
- Techniques for Introducing and Modifying Disulfide Bonds
- Computational Tools and Predictive Modeling in Bond Design
- Case Studies: Enhanced Protein Stability and Function
- Applications in Therapeutic Protein and Enzyme Development
- Challenges and Limitations in Disulfide Bond Engineering
- Emerging Technologies: CRISPR, AI, and Synthetic Biology Integration
- Market Trends and Public Interest: Growth Forecasts and Industry Impact
- Future Outlook: Innovations and Opportunities in Protein Engineering
- Sources & References
Introduction: The Role of Disulfide Bonds in Protein Structure
Disulfide bonds are covalent linkages formed between the thiol groups of cysteine residues within or between polypeptide chains. These bonds play a pivotal role in stabilizing the three-dimensional structure of proteins, particularly those secreted into oxidizing environments such as the extracellular space. The formation of disulfide bonds contributes to the rigidity and resilience of protein structures, enhancing their resistance to denaturation and proteolytic degradation. This structural reinforcement is especially critical for proteins that function under harsh physiological or industrial conditions.
In the context of protein design, disulfide bond engineering refers to the deliberate introduction, removal, or rearrangement of disulfide linkages to modulate protein stability, folding, and function. By strategically engineering these covalent bonds, researchers can improve the thermal and chemical stability of proteins, optimize their folding pathways, and even alter their biological activity. This approach is widely utilized in the development of therapeutic proteins, industrial enzymes, and novel biomaterials.
The significance of disulfide bonds in protein architecture is underscored by their prevalence in a variety of biologically important molecules, including antibodies, hormones, and enzymes. For example, the immunoglobulin fold, a common structural motif in antibodies, is stabilized by conserved disulfide bridges that are essential for antigen recognition and immune response. Similarly, many enzymes rely on disulfide bonds to maintain their active conformations and catalytic efficiency.
Advances in computational modeling and synthetic biology have greatly facilitated the rational design of disulfide bonds in proteins. Modern techniques allow for the prediction of optimal cysteine pairings and the assessment of their impact on protein structure and function. These innovations are supported by organizations such as the Research Collaboratory for Structural Bioinformatics (RCSB), which maintains the Protein Data Bank (PDB), a comprehensive resource for protein structural data. The National Institute of General Medical Sciences (NIGMS), a division of the U.S. National Institutes of Health, also funds research into protein structure and engineering, highlighting the importance of disulfide bonds in biomedical science.
As the field of protein engineering continues to evolve, the manipulation of disulfide bonds remains a cornerstone strategy for enhancing protein performance and expanding their applications in medicine, industry, and research. Understanding the fundamental role of these covalent linkages is essential for the rational design of next-generation proteins with tailored properties.
Historical Milestones in Disulfide Bond Engineering
Disulfide bond engineering has played a pivotal role in the evolution of protein design, enabling the stabilization and functional optimization of proteins for diverse applications. The historical trajectory of this field is marked by several key milestones that have shaped current methodologies and understanding.
The foundational discovery of disulfide bonds in proteins dates back to the early 20th century, when researchers first identified the covalent linkage between cysteine residues as a critical determinant of protein structure and stability. The classic work of Frederick Sanger in the 1950s, which elucidated the structure of insulin and its disulfide bridges, provided the first concrete evidence of the importance of these bonds in maintaining protein conformation. This breakthrough laid the groundwork for subsequent efforts to manipulate disulfide bonds for protein engineering purposes.
In the 1980s and 1990s, advances in recombinant DNA technology and site-directed mutagenesis enabled scientists to introduce or remove cysteine residues at specific locations within protein sequences. This era saw the first deliberate engineering of disulfide bonds to enhance protein stability, as demonstrated in studies on enzymes and antibodies. The ability to rationally design disulfide bridges allowed researchers to improve the thermal and chemical resilience of proteins, expanding their utility in industrial and therapeutic contexts.
A significant milestone was the development of computational tools for predicting disulfide bond formation and stability. The integration of structural bioinformatics and molecular modeling in the late 1990s and early 2000s facilitated the identification of optimal sites for disulfide engineering, reducing the reliance on trial-and-error approaches. Organizations such as the Research Collaboratory for Structural Bioinformatics (RCSB), which maintains the Protein Data Bank, have been instrumental in providing structural data that underpin these computational advances.
More recently, the advent of synthetic biology and high-throughput screening has accelerated the pace of disulfide bond engineering. Automated platforms now enable the rapid generation and evaluation of protein variants with engineered disulfide bonds, streamlining the discovery of robust protein scaffolds for pharmaceuticals, diagnostics, and industrial enzymes. The National Institute of General Medical Sciences (NIGMS), a part of the U.S. National Institutes of Health, has supported research initiatives that advance the understanding and application of disulfide engineering in protein science.
As of 2025, disulfide bond engineering stands as a mature and continually evolving discipline, with historical milestones reflecting the interplay of biochemical insight, technological innovation, and collaborative research efforts. These achievements have not only deepened our understanding of protein structure but have also enabled the rational design of proteins with tailored properties for a wide range of scientific and medical applications.
Techniques for Introducing and Modifying Disulfide Bonds
Disulfide bond engineering is a pivotal strategy in protein design, enabling the stabilization, functional modulation, and structural refinement of proteins for diverse applications in biotechnology, therapeutics, and industrial catalysis. The introduction and modification of disulfide bonds—covalent linkages formed between the thiol groups of cysteine residues—require a combination of computational, genetic, and chemical techniques to ensure precise placement and optimal protein performance.
One of the foundational approaches involves site-directed mutagenesis, where specific amino acid residues are substituted with cysteines at positions predicted to favor disulfide bond formation. This method relies on detailed structural analysis, often guided by high-resolution protein models or X-ray crystallography data, to identify candidate sites that are spatially proximate and oriented favorably for bond formation. Computational tools, such as molecular dynamics simulations and disulfide bond prediction algorithms, further refine these choices by assessing the energetic feasibility and potential impact on protein folding and function.
Advances in synthetic biology have expanded the toolkit for disulfide bond engineering. Techniques such as gene synthesis and codon optimization allow for the rapid generation of protein variants with engineered cysteine pairs. In parallel, directed evolution approaches—where libraries of protein variants are screened for enhanced stability or activity—can identify beneficial disulfide bonds that may not be apparent from rational design alone. These methods are often supported by high-throughput screening platforms and automated protein expression systems.
Chemical methods also play a role in disulfide bond modification. In vitro oxidation using mild oxidizing agents can facilitate the formation of disulfide bonds in recombinant proteins expressed in reducing environments, such as the cytoplasm of Escherichia coli. Additionally, chemical cross-linkers that mimic disulfide bonds can be employed to stabilize protein structures when natural cysteine placement is not feasible.
The choice of host organism for protein expression is another critical consideration. Eukaryotic systems, such as yeast or mammalian cells, possess the cellular machinery for correct disulfide bond formation and isomerization, while prokaryotic systems may require co-expression of folding catalysts like protein disulfide isomerase. Organizations such as the National Institutes of Health and the European Molecular Biology Laboratory provide resources and guidelines for best practices in protein engineering and expression.
In summary, the successful engineering of disulfide bonds in proteins is achieved through a synergistic application of computational design, genetic manipulation, chemical modification, and optimized expression systems. These techniques collectively enable the rational and efficient enhancement of protein stability and function, supporting advances in research and biotechnological innovation.
Computational Tools and Predictive Modeling in Bond Design
Disulfide bond engineering has become a cornerstone in the rational design of proteins, offering a means to enhance protein stability, modulate function, and improve therapeutic properties. The advent of advanced computational tools and predictive modeling has significantly accelerated the identification and optimization of disulfide bonds in protein structures. These approaches leverage structural bioinformatics, molecular dynamics simulations, and machine learning algorithms to predict the most favorable sites for disulfide bond introduction, minimizing experimental trial-and-error and reducing development timelines.
A primary step in computational disulfide bond engineering involves the analysis of protein three-dimensional structures to identify residue pairs that are spatially proximate and geometrically compatible for disulfide linkage. Tools such as Disulfide by Design and MODIP (Modeling Disulfide Bonds in Proteins) automate this process, evaluating geometric criteria like Cβ–Cβ distances and dihedral angles to suggest candidate sites. These platforms often integrate with structural databases maintained by organizations such as the RCSB Protein Data Bank, which provides high-resolution protein structures essential for accurate modeling.
Beyond static structure analysis, molecular dynamics (MD) simulations are employed to assess the dynamic behavior of engineered disulfide bonds under physiological conditions. MD simulations, facilitated by software like GROMACS and AMBER, allow researchers to predict the impact of disulfide introduction on protein flexibility, folding pathways, and overall stability. These simulations are critical for anticipating potential destabilizing effects or unintended conformational changes that may arise from new covalent linkages.
Recent advances in artificial intelligence and machine learning have further refined predictive modeling in disulfide bond engineering. Deep learning models, trained on large datasets of protein structures and mutational outcomes, can forecast the thermodynamic and functional consequences of specific disulfide bond insertions. Initiatives by organizations such as DeepMind—notably through the development of AlphaFold—have revolutionized protein structure prediction, enabling more accurate in silico design of disulfide bonds even in proteins lacking experimental structures.
The integration of these computational tools into protein engineering workflows has been endorsed by leading scientific bodies, including the Nature Publishing Group and the National Institute of General Medical Sciences, which highlight their role in expediting the rational design of stable and functional proteins. As computational power and algorithmic sophistication continue to advance, predictive modeling is expected to play an increasingly central role in the future of disulfide bond engineering.
Case Studies: Enhanced Protein Stability and Function
Disulfide bond engineering has emerged as a pivotal strategy in protein design, enabling the enhancement of protein stability and function through the rational introduction or modification of disulfide bridges. Disulfide bonds, covalent linkages formed between the thiol groups of cysteine residues, play a crucial role in stabilizing the tertiary and quaternary structures of proteins. By leveraging advances in computational modeling and structural biology, researchers have successfully engineered novel disulfide bonds to improve protein robustness, resistance to denaturation, and functional performance in diverse applications.
One notable case study involves the engineering of disulfide bonds in industrial enzymes to increase their thermal and chemical stability. For example, the introduction of additional disulfide bridges in subtilisin, a widely used protease, resulted in variants with significantly enhanced resistance to heat and detergents, thereby extending their utility in harsh industrial processes. This approach has been replicated in other enzymes, such as lipases and cellulases, where engineered disulfide bonds have led to improved operational stability and prolonged shelf life, directly benefiting sectors like biofuels and detergents.
In the field of therapeutic proteins, disulfide bond engineering has been instrumental in optimizing antibody fragments and cytokines. For instance, the stabilization of single-chain variable fragments (scFvs) through engineered disulfide bonds has yielded molecules with greater conformational stability and reduced aggregation, which are critical attributes for clinical development and manufacturing. Similarly, cytokines engineered with novel disulfide linkages have demonstrated enhanced in vivo half-life and bioactivity, supporting their use in immunotherapy and other medical applications.
A prominent example from vaccine development is the stabilization of viral glycoproteins through disulfide bond engineering. The prefusion conformation of viral fusion proteins, such as those from respiratory syncytial virus (RSV) and coronaviruses, is often metastable. By introducing strategic disulfide bonds, researchers have successfully locked these proteins in their immunogenic prefusion states, leading to improved vaccine efficacy. This strategy was notably applied in the design of stabilized spike proteins for SARS-CoV-2 vaccines, contributing to the rapid development of effective immunogens.
These case studies underscore the transformative impact of disulfide bond engineering in protein design, offering robust solutions to challenges in stability and function. The continued integration of computational design tools, high-throughput screening, and structural analysis is expected to further expand the repertoire of engineered proteins with tailored properties for industrial, therapeutic, and research applications. Leading organizations such as the National Institutes of Health and the European Molecular Biology Laboratory actively support and disseminate research in this domain, fostering innovation and collaboration across the global scientific community.
Applications in Therapeutic Protein and Enzyme Development
Disulfide bond engineering has emerged as a pivotal strategy in the design and optimization of therapeutic proteins and enzymes, offering enhanced stability, activity, and manufacturability. Disulfide bonds, covalent linkages formed between the thiol groups of cysteine residues, play a crucial role in stabilizing the three-dimensional structure of proteins. By introducing, removing, or rearranging these bonds, researchers can tailor protein properties to meet specific therapeutic or industrial requirements.
In therapeutic protein development, disulfide bond engineering is frequently employed to improve the stability and shelf-life of biopharmaceuticals, such as monoclonal antibodies, hormones, and cytokines. Enhanced stability is particularly important for proteins administered in challenging physiological environments or requiring long-term storage. For example, the rational introduction of additional disulfide bonds has been shown to increase the thermal and proteolytic stability of antibody fragments, thereby improving their pharmacokinetic profiles and reducing the frequency of dosing. This approach is also used to minimize aggregation and misfolding, common issues that can compromise the efficacy and safety of protein therapeutics.
Enzyme engineering also benefits significantly from disulfide bond manipulation. Industrial enzymes, which often operate under harsh conditions such as extreme pH or high temperatures, require robust structural integrity. By strategically engineering new disulfide bonds, scientists can enhance enzyme resilience, prolonging their functional lifespan and expanding their utility in diverse applications, including biocatalysis, food processing, and biofuel production. For instance, the introduction of non-native disulfide bonds has been demonstrated to increase the thermostability of enzymes like lipases and proteases, making them more suitable for industrial-scale processes.
The design and implementation of disulfide bonds in proteins rely on advanced computational modeling and structural biology techniques. Organizations such as the Research Collaboratory for Structural Bioinformatics (RCSB), which maintains the Protein Data Bank, provide critical structural data that inform rational engineering strategies. Additionally, regulatory bodies like the U.S. Food and Drug Administration (FDA) oversee the development and approval of engineered therapeutic proteins, ensuring their safety and efficacy for clinical use.
As the demand for more effective and stable biotherapeutics and industrial enzymes continues to grow, disulfide bond engineering is expected to play an increasingly central role in protein design. Ongoing advances in computational protein design, high-throughput screening, and synthetic biology are poised to further expand the possibilities for precise and efficient disulfide bond manipulation in the coming years.
Challenges and Limitations in Disulfide Bond Engineering
Disulfide bond engineering has emerged as a powerful strategy in protein design, enabling the stabilization of protein structures, enhancement of thermal and chemical stability, and improvement of therapeutic protein properties. However, despite its promise, several challenges and limitations persist, particularly as the field advances into 2025.
One of the primary challenges lies in the accurate prediction of disulfide bond formation and its impact on protein folding. The introduction of new disulfide bonds can inadvertently disrupt native folding pathways, leading to misfolded or aggregated proteins. This is especially problematic in complex or multi-domain proteins, where the spatial proximity of cysteine residues does not always guarantee successful bond formation. Computational tools have improved, but the dynamic nature of protein folding and the influence of the cellular environment still limit predictive accuracy.
Another significant limitation is the cellular machinery required for correct disulfide bond formation. In prokaryotic expression systems such as Escherichia coli, the reducing environment of the cytoplasm is not conducive to disulfide bond formation, often necessitating the use of specialized strains or expression in the periplasm. Even in eukaryotic systems, the efficiency of disulfide bond formation can be hampered by the availability of protein disulfide isomerases and the oxidative folding environment. This can result in heterogeneous products and reduced yields, complicating downstream processing and scalability.
Immunogenicity is another concern, particularly for therapeutic proteins. The introduction of non-native disulfide bonds can create novel epitopes, potentially triggering unwanted immune responses in patients. Regulatory agencies such as the U.S. Food and Drug Administration and the European Medicines Agency require rigorous characterization of engineered proteins to assess such risks, adding complexity to the development pipeline.
Furthermore, the functional consequences of disulfide bond engineering are not always predictable. While the goal is often to enhance stability, new disulfide bonds can restrict necessary conformational flexibility, impairing protein function or activity. This is particularly relevant for enzymes and signaling proteins, where dynamic structural changes are integral to their biological roles.
Finally, the field faces technical limitations in high-throughput screening and validation of engineered proteins. Although advances in synthetic biology and analytical techniques have accelerated progress, the iterative process of design, expression, and characterization remains resource-intensive.
In summary, while disulfide bond engineering holds significant potential for advancing protein design, overcoming these challenges will require continued innovation in computational modeling, expression technologies, and regulatory science, as recognized by leading organizations such as the National Institute of General Medical Sciences and the National Science Foundation.
Emerging Technologies: CRISPR, AI, and Synthetic Biology Integration
Disulfide bond engineering has emerged as a pivotal strategy in protein design, offering enhanced stability, functionality, and therapeutic potential for engineered proteins. The integration of emerging technologies such as CRISPR-based genome editing, artificial intelligence (AI), and synthetic biology is accelerating advances in this field, particularly as researchers seek to design proteins with tailored disulfide patterns for industrial, medical, and research applications.
Disulfide bonds, covalent linkages between cysteine residues, play a crucial role in stabilizing the three-dimensional structure of proteins. Rational engineering of these bonds can improve protein folding, resistance to denaturation, and overall bioactivity. Traditional approaches relied on labor-intensive mutagenesis and screening; however, the advent of AI-driven protein modeling and prediction tools has revolutionized the identification of optimal sites for disulfide introduction. AI algorithms, such as those developed by leading research institutions and technology companies, can predict the effects of disulfide bond insertion on protein structure and function with unprecedented accuracy, reducing experimental workload and accelerating discovery.
CRISPR-Cas genome editing technologies further enable precise manipulation of genetic sequences encoding target proteins. By introducing or modifying cysteine codons at specific locations, researchers can program the formation of new disulfide bonds in vivo. This approach is particularly valuable in the context of therapeutic protein production, where enhanced stability and reduced immunogenicity are critical. Organizations such as Broad Institute and National Institutes of Health have been at the forefront of developing and disseminating CRISPR-based methodologies for protein engineering.
Synthetic biology platforms provide modular toolkits for assembling and expressing engineered proteins with customized disulfide architectures. These platforms leverage standardized genetic parts, automated DNA synthesis, and high-throughput screening to streamline the design-build-test cycle. The integration of AI with synthetic biology enables the rapid prototyping of protein variants, while CRISPR ensures precise genomic integration and expression control. Notably, organizations such as SynBioBeta and European Molecular Biology Laboratory (EMBL) are actively promoting the convergence of these technologies to advance protein engineering.
Looking ahead to 2025, the synergy between CRISPR, AI, and synthetic biology is expected to further democratize and accelerate disulfide bond engineering. This will facilitate the development of next-generation biotherapeutics, industrial enzymes, and novel biomaterials with enhanced performance and stability, underscoring the transformative potential of these integrated approaches in protein design.
Market Trends and Public Interest: Growth Forecasts and Industry Impact
Disulfide bond engineering has emerged as a pivotal strategy in protein design, with significant implications for the biotechnology, pharmaceutical, and industrial enzyme sectors. As the demand for stable, functional, and tailor-made proteins continues to rise, the market for disulfide bond engineering is experiencing robust growth. This trend is driven by the increasing adoption of protein-based therapeutics, the expansion of enzyme applications in green chemistry, and the need for improved biopharmaceutical stability and efficacy.
Forecasts for 2025 indicate that the global protein engineering market, within which disulfide bond engineering is a key technology, will continue its upward trajectory. The integration of advanced computational tools, such as artificial intelligence and machine learning, is accelerating the rational design of disulfide bonds, enabling the creation of proteins with enhanced thermal stability, resistance to proteolysis, and optimized activity profiles. These advances are particularly relevant for therapeutic proteins, where disulfide bonds can improve pharmacokinetics and reduce immunogenicity, and for industrial enzymes, where stability under harsh conditions is essential.
Major industry players and research organizations are investing heavily in the development and commercialization of disulfide bond engineering technologies. For example, Genentech, a pioneer in protein therapeutics, and Amgen, a leader in biopharmaceutical innovation, are actively exploring disulfide engineering to enhance the stability and manufacturability of their protein-based drugs. Additionally, organizations such as the National Institute of General Medical Sciences (NIGMS), part of the U.S. National Institutes of Health, support fundamental research into protein structure and engineering, including the role of disulfide bonds in protein folding and function.
Public interest in disulfide bond engineering is also growing, fueled by the promise of more effective biologic drugs, sustainable industrial processes, and novel biomaterials. The technology’s potential to address challenges in protein misfolding diseases, such as cystic fibrosis and certain cancers, further amplifies its societal impact. As regulatory agencies like the U.S. Food and Drug Administration (FDA) continue to approve an increasing number of protein-based therapeutics, the importance of robust protein engineering methods, including disulfide bond manipulation, is expected to rise.
In summary, the market for disulfide bond engineering in protein design is poised for significant growth in 2025 and beyond, driven by technological innovation, industry investment, and expanding applications across healthcare and industry. The convergence of scientific advances and public interest underscores the transformative potential of this field.
Future Outlook: Innovations and Opportunities in Protein Engineering
Disulfide bond engineering is poised to play a transformative role in the future of protein engineering, offering innovative solutions to longstanding challenges in protein stability, function, and therapeutic application. Disulfide bonds, covalent linkages formed between cysteine residues, are critical for maintaining the structural integrity and biological activity of many proteins. By strategically introducing or modifying these bonds, researchers can enhance protein folding, increase resistance to denaturation, and fine-tune functional properties, which is particularly valuable in the design of biopharmaceuticals, industrial enzymes, and novel biomaterials.
Recent advances in computational modeling and high-throughput screening are accelerating the rational design of disulfide bonds. Machine learning algorithms and molecular dynamics simulations now enable the prediction of optimal sites for disulfide introduction, minimizing the risk of misfolding or aggregation. These computational tools are being integrated into protein engineering pipelines, allowing for the rapid prototyping and optimization of disulfide-stabilized proteins. Organizations such as the National Institutes of Health and the European Molecular Biology Laboratory are at the forefront of supporting research in this area, funding projects that leverage artificial intelligence to design more robust and functional proteins.
In the therapeutic realm, disulfide bond engineering is opening new avenues for the development of next-generation biologics. For example, monoclonal antibodies and other protein-based drugs can be engineered with additional or reshaped disulfide bonds to improve their stability in the bloodstream, reduce immunogenicity, and extend shelf life. This is particularly relevant for the development of biosimilars and biobetters, where enhanced stability can translate into improved patient outcomes and reduced manufacturing costs. Regulatory agencies such as the U.S. Food and Drug Administration are increasingly evaluating the impact of such modifications on drug safety and efficacy, underscoring the importance of rigorous characterization and validation.
Looking ahead to 2025 and beyond, the integration of disulfide bond engineering with emerging technologies—such as synthetic biology, directed evolution, and CRISPR-based genome editing—will further expand the possibilities for custom-designed proteins. These innovations are expected to drive the creation of novel therapeutics, environmentally resilient enzymes for industrial processes, and advanced biomaterials with tailored mechanical and chemical properties. As the field continues to evolve, collaboration between academic institutions, government agencies, and industry leaders will be essential to realize the full potential of disulfide bond engineering in protein design.
Sources & References
- Research Collaboratory for Structural Bioinformatics
- National Institute of General Medical Sciences
- National Institutes of Health
- European Molecular Biology Laboratory
- DeepMind
- Nature Publishing Group
- European Medicines Agency
- National Science Foundation
- Broad Institute
- SynBioBeta