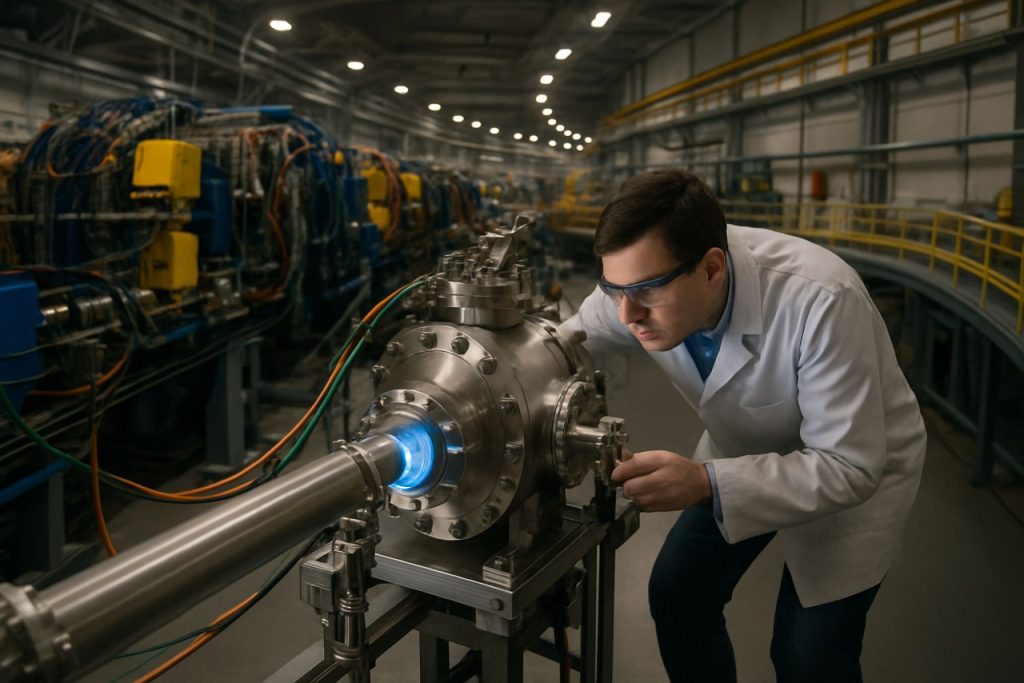
Synchrotron Radiation Explained: How Ultra-Bright Beams Are Revolutionizing Science and Technology. Discover the Secrets Behind the World’s Most Advanced Light Source.
- Introduction to Synchrotron Radiation
- The Physics Behind Synchrotron Light
- Historical Development and Milestones
- Modern Synchrotron Facilities Around the World
- Applications in Science and Industry
- Medical and Biological Breakthroughs Enabled by Synchrotron Radiation
- Technological Innovations and Instrumentation
- Challenges and Future Directions
- Conclusion: The Expanding Frontier of Synchrotron Research
- Sources & References
Introduction to Synchrotron Radiation
Synchrotron radiation is a highly intense, broad-spectrum electromagnetic radiation emitted when charged particles, such as electrons, are accelerated to relativistic speeds and forced to travel along curved paths by magnetic fields. First observed in 1947 at the General Electric synchrotron, this phenomenon has since become a cornerstone of modern scientific research, enabling breakthroughs across physics, chemistry, biology, and materials science. Unlike conventional light sources, synchrotron radiation is characterized by its exceptional brightness, tunability across a wide range of wavelengths (from infrared to hard X-rays), and high degree of polarization, making it uniquely suited for probing the structure and properties of matter at atomic and molecular scales.
Modern synchrotron facilities, often referred to as “light sources,” use large storage rings to accelerate electrons to near-light speeds. As these electrons are deflected by powerful magnets, they emit synchrotron radiation tangentially to their path. The resulting beams are extracted and directed to experimental stations, where they are used for a variety of advanced techniques, including X-ray diffraction, spectroscopy, and imaging. These capabilities have revolutionized fields such as structural biology—enabling the determination of complex protein structures—and materials science, where they facilitate the study of nanomaterials and electronic devices.
The global network of synchrotron facilities, such as the European Synchrotron Radiation Facility and the Advanced Photon Source, continues to expand, driving innovation and collaboration across disciplines. As technology advances, synchrotron radiation remains an indispensable tool for exploring the frontiers of science and technology.
The Physics Behind Synchrotron Light
Synchrotron light is produced when charged particles, typically electrons, are accelerated to relativistic speeds and forced to travel along curved paths by strong magnetic fields. This process is governed by the principles of classical electrodynamics and special relativity. As electrons traverse a circular or spiral trajectory within a synchrotron or storage ring, they experience centripetal acceleration, which causes them to emit electromagnetic radiation tangentially to their path. The emitted radiation spans a broad spectrum, from infrared to hard X-rays, and is characterized by its high brightness, collimation, and polarization.
The underlying physics can be described by the Liénard–Wiechert potentials, which account for the relativistic motion of the electrons. The intensity and angular distribution of the emitted synchrotron radiation depend on the electron energy and the strength of the magnetic field. At relativistic speeds, the radiation is highly collimated in the forward direction due to Lorentz contraction, resulting in a narrow emission cone. The spectral properties are also influenced by the critical energy, which marks the photon energy at which the power spectrum of the emitted radiation peaks. This critical energy increases with both the electron energy and the magnetic field strength, allowing synchrotron facilities to tailor the output for specific experimental needs.
The unique properties of synchrotron light—such as its tunable wavelength, high intensity, and polarization—make it an invaluable tool for probing the structure of matter at atomic and molecular scales. For a detailed explanation of the physics and applications, see resources from the European Synchrotron Radiation Facility and the Brookhaven National Laboratory.
Historical Development and Milestones
The historical development of synchrotron radiation traces back to the mid-20th century, marking a significant milestone in both physics and technology. The phenomenon was first observed in 1947 at the General Electric Research Laboratory, where researchers noticed an unexpected bright blue light emitted from electrons circulating in a synchrotron accelerator. This discovery, initially considered a nuisance due to energy loss, soon revealed profound scientific potential. Early theoretical groundwork had been laid by physicists such as Julian Schwinger, who provided a comprehensive quantum mechanical description of the radiation process.
Throughout the 1950s and 1960s, synchrotron radiation was primarily a byproduct of particle physics experiments. However, its unique properties—high brightness, broad spectral range, and polarization—quickly attracted the attention of researchers in other fields. The first dedicated synchrotron light source, the Synchrotron Radiation Source (SRS) at Daresbury Laboratory in the UK, became operational in 1980, marking a pivotal shift from parasitic to purpose-built facilities. This transition enabled the development of specialized beamlines and instrumentation, greatly expanding the range of scientific applications.
Subsequent decades saw the construction of increasingly advanced synchrotron facilities worldwide, such as the European Synchrotron Radiation Facility (European Synchrotron Radiation Facility) and the Advanced Photon Source (Argonne National Laboratory). These milestones have established synchrotron radiation as an indispensable tool in fields ranging from materials science to biology, catalyzing breakthroughs in protein crystallography, nanotechnology, and environmental science. The ongoing evolution of synchrotron technology continues to push the boundaries of scientific discovery.
Modern Synchrotron Facilities Around the World
Modern synchrotron facilities represent the pinnacle of large-scale scientific infrastructure, enabling cutting-edge research across physics, chemistry, biology, materials science, and environmental studies. These facilities house advanced storage rings and beamlines that generate and manipulate synchrotron radiation—intense, highly collimated beams of X-rays and other electromagnetic radiation. Today, more than 50 synchrotron light sources operate globally, each with unique capabilities and user communities.
Among the most prominent is the European Synchrotron Radiation Facility (ESRF) in France, which recently underwent a major upgrade to its Extremely Brilliant Source, offering unprecedented brightness and coherence. In the United States, the National Synchrotron Light Source II (NSLS-II) at Brookhaven National Laboratory provides ultra-high brightness X-rays for nanoscale imaging and spectroscopy. Asia is home to several world-class facilities, including Japan’s SPring-8, renowned for its high-energy X-ray capabilities, and China’s Shanghai Synchrotron Radiation Facility (SSRF), which supports a rapidly growing scientific community.
These facilities are continually evolving, with new generations of synchrotrons—so-called “diffraction-limited storage rings”—pushing the boundaries of spatial and temporal resolution. International collaboration is a hallmark of the field, with researchers from around the world accessing beamtime through competitive proposal systems. The global network of synchrotron facilities not only accelerates scientific discovery but also fosters innovation in medicine, energy, and technology, underscoring the central role of synchrotron radiation in modern science.
Applications in Science and Industry
Synchrotron radiation has become an indispensable tool across a wide spectrum of scientific and industrial fields due to its unique properties: high brightness, broad spectral range (from infrared to hard X-rays), and tunable polarization. In materials science, synchrotron light enables detailed structural analysis at the atomic and molecular levels, facilitating the development of advanced materials and nanotechnologies. Techniques such as X-ray diffraction and X-ray absorption spectroscopy, performed at synchrotron facilities, allow researchers to probe the arrangement of atoms and electronic states in complex materials, leading to breakthroughs in electronics, energy storage, and catalysis (European Synchrotron Radiation Facility).
In the life sciences, synchrotron radiation is crucial for determining the three-dimensional structures of biological macromolecules through macromolecular crystallography. This has accelerated drug discovery and the understanding of fundamental biological processes. Medical imaging also benefits from synchrotron-based techniques, such as phase-contrast imaging, which provides higher resolution and contrast than conventional X-ray methods (Diamond Light Source).
Industrial applications include non-destructive testing and quality control, where synchrotron X-rays can reveal internal defects in manufactured components with exceptional precision. The semiconductor industry utilizes synchrotron radiation for lithography and the analysis of microelectronic devices. Environmental science also leverages synchrotron techniques to study pollutants at trace levels and their interactions with natural materials (Australian Synchrotron).
Overall, the versatility and power of synchrotron radiation continue to drive innovation and discovery across disciplines, making it a cornerstone of modern scientific and industrial research.
Medical and Biological Breakthroughs Enabled by Synchrotron Radiation
Synchrotron radiation has revolutionized medical and biological research by providing exceptionally bright, tunable X-ray beams that enable high-resolution imaging and structural analysis at the molecular and cellular levels. One of the most significant breakthroughs is in macromolecular crystallography, where synchrotron sources have allowed scientists to determine the three-dimensional structures of complex proteins, viruses, and nucleic acids with unprecedented detail. This structural information is crucial for rational drug design, as it enables researchers to understand the precise interactions between drugs and their biological targets, accelerating the development of new therapeutics for diseases such as cancer, HIV/AIDS, and neurodegenerative disorders (EMBL Hamburg).
In addition to structural biology, synchrotron radiation has advanced imaging techniques such as phase-contrast X-ray imaging and X-ray fluorescence microscopy. These methods allow for non-destructive, high-contrast visualization of soft tissues, cells, and even subcellular components, which are often invisible with conventional X-ray sources. Such capabilities have led to breakthroughs in understanding the progression of diseases like osteoporosis, cancer, and cardiovascular conditions, as well as in mapping the distribution of trace elements in biological tissues (Diamond Light Source).
Furthermore, synchrotron-based infrared and X-ray spectroscopies have enabled real-time studies of biochemical processes, such as enzyme catalysis and drug metabolism, under physiological conditions. These insights are invaluable for both basic biological research and the development of medical diagnostics and treatments (European Synchrotron Radiation Facility).
Technological Innovations and Instrumentation
Technological innovations in synchrotron radiation facilities have dramatically expanded the capabilities and applications of this powerful scientific tool. Modern synchrotrons employ advanced storage ring designs, such as multi-bend achromat lattices, which significantly enhance beam brightness and coherence. These improvements enable researchers to probe matter at unprecedented spatial and temporal resolutions, facilitating breakthroughs in fields ranging from structural biology to materials science. For instance, the implementation of European Synchrotron Radiation Facility‘s Extremely Brilliant Source (EBS) upgrade has resulted in a 100-fold increase in X-ray brilliance, allowing for more detailed imaging and faster data collection.
Instrumentation associated with synchrotron radiation has also evolved rapidly. State-of-the-art beamlines are now equipped with high-precision monochromators, adaptive optics, and fast detectors capable of handling high photon fluxes and delivering real-time data. Innovations such as cryogenic sample environments, micro- and nano-focusing optics, and automated sample changers have further enhanced experimental throughput and reproducibility. Additionally, the integration of advanced computing and machine learning algorithms enables efficient data analysis and experiment optimization, as demonstrated by initiatives at the Advanced Photon Source and SLAC National Accelerator Laboratory.
These technological advancements not only improve the quality and scope of synchrotron-based research but also make the facilities more accessible to a broader scientific community. As a result, synchrotron radiation continues to drive innovation across diverse disciplines, supporting both fundamental research and industrial applications.
Challenges and Future Directions
Despite its transformative impact on fields ranging from materials science to biology, synchrotron radiation research faces several challenges that shape its future trajectory. One major challenge is the escalating demand for higher brightness and coherence, which necessitates continual upgrades to existing facilities and the construction of next-generation light sources, such as diffraction-limited storage rings and free-electron lasers. These advancements require significant financial investment and technical innovation, particularly in accelerator technology and beamline instrumentation European Synchrotron Radiation Facility.
Another challenge lies in data management. The increasing brilliance and speed of modern synchrotrons generate vast volumes of complex data, demanding robust computational infrastructure and advanced algorithms for real-time analysis and storage. This has spurred collaborations between synchrotron facilities and data science experts to develop new tools for data processing and machine learning applications Paul Scherrer Institute.
Looking ahead, future directions include the miniaturization and democratization of synchrotron sources, with the development of compact, laboratory-scale devices that could make advanced X-ray techniques more widely accessible Lightsources.org. Additionally, there is a growing emphasis on sustainability, with facilities exploring energy-efficient operation and green technologies. Interdisciplinary research, integrating synchrotron radiation with complementary methods such as cryo-electron microscopy and neutron scattering, is also expected to expand, further enhancing the scientific reach and societal impact of synchrotron science.
Conclusion: The Expanding Frontier of Synchrotron Research
The field of synchrotron radiation research continues to expand, driven by advances in accelerator technology, detector sensitivity, and computational analysis. Modern synchrotron facilities, such as those operated by the European Synchrotron Radiation Facility and the Advanced Photon Source, provide scientists with unprecedented access to high-brilliance, tunable X-ray beams. These capabilities have enabled breakthroughs across disciplines, from structural biology and materials science to environmental studies and cultural heritage preservation. The ability to probe matter at atomic and molecular scales has led to the development of new pharmaceuticals, advanced materials, and a deeper understanding of fundamental processes in chemistry and physics.
Looking ahead, the frontier of synchrotron research is being pushed further by the advent of fourth-generation light sources, such as diffraction-limited storage rings and X-ray free-electron lasers. These innovations promise even higher spatial and temporal resolution, opening new possibilities for observing ultrafast phenomena and transient states of matter. Collaborative international initiatives, exemplified by the Lightsources.org consortium, are fostering knowledge exchange and resource sharing, accelerating scientific progress worldwide. As synchrotron radiation techniques become more accessible and versatile, their impact is expected to grow, supporting innovation in energy, health, nanotechnology, and beyond. The expanding frontier of synchrotron research thus represents a vital and dynamic domain at the intersection of science and technology.
Sources & References
- European Synchrotron Radiation Facility
- Advanced Photon Source
- Brookhaven National Laboratory
- Australian Synchrotron
- Paul Scherrer Institute
- Lightsources.org