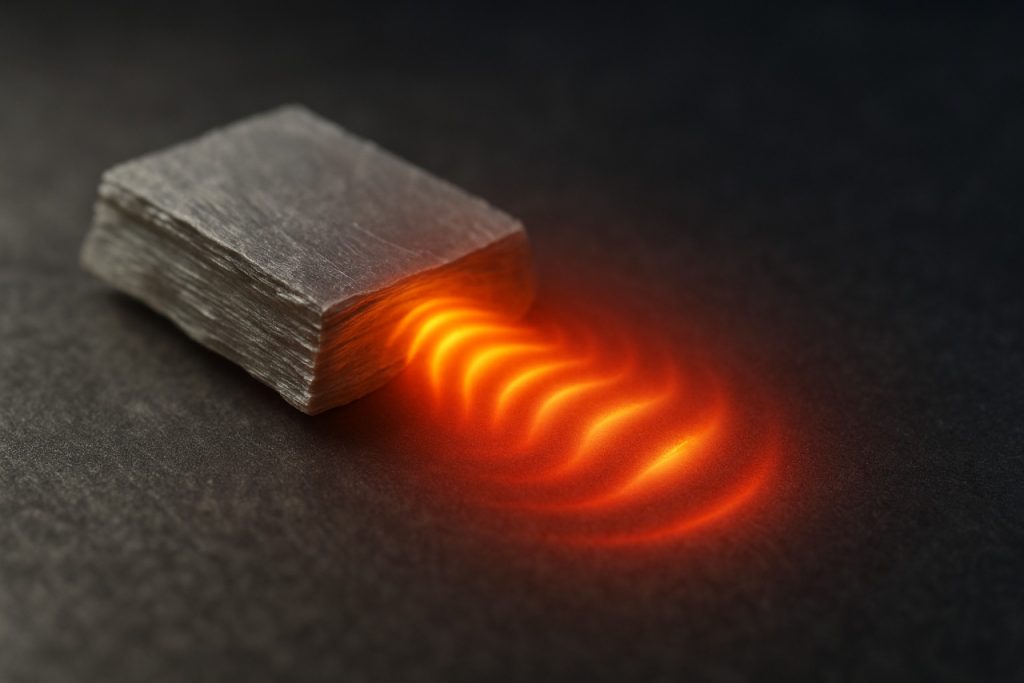
Phonon Polaritons in Hexagonal Boron Nitride: Revolutionizing Nanoscale Light-Matter Interactions. Discover How hBN Enables Unprecedented Control Over Mid-Infrared Photonics.
- Introduction to Phonon Polaritons and Hexagonal Boron Nitride
- Fundamental Physics of Phonon Polaritons in hBN
- Crystal Structure and Optical Properties of hBN
- Hyperbolic Dispersion and Anisotropy in hBN
- Experimental Techniques for Probing Phonon Polaritons
- Theoretical Models and Computational Approaches
- Applications in Nanoscale Photonics and Sensing
- Integration with Other 2D Materials and Heterostructures
- Challenges and Limitations in Current Research
- Future Directions and Emerging Opportunities
- Sources & References
Introduction to Phonon Polaritons and Hexagonal Boron Nitride
Phonon polaritons are hybrid quasiparticles that emerge from the strong coupling between photons and optical phonons within polar dielectric materials. These excitations enable the confinement and manipulation of electromagnetic energy at subwavelength scales, far below the diffraction limit of light. The study of phonon polaritons has garnered significant attention due to their potential applications in nanophotonics, infrared (IR) optics, and quantum technologies. Among the various materials supporting phonon polaritons, hexagonal boron nitride (hBN) stands out as a particularly promising platform.
Hexagonal boron nitride is a two-dimensional (2D) van der Waals crystal composed of alternating boron and nitrogen atoms arranged in a hexagonal lattice. Its structure is analogous to that of graphene, but with a wide bandgap and strong ionic character. This unique combination of properties imparts hBN with exceptional thermal stability, chemical inertness, and a high degree of optical anisotropy. Most notably, hBN exhibits two distinct Reststrahlen bands—frequency ranges in the mid-infrared where its dielectric permittivity is negative—enabling the propagation of phonon polaritons with hyperbolic dispersion. In these bands, hBN supports highly confined, low-loss polaritonic modes that can be tuned by varying the thickness or geometry of the material.
The ability of hBN to support phonon polaritons arises from its polar lattice vibrations, specifically the optical phonons associated with the relative motion of boron and nitrogen atoms. When mid-infrared light interacts with hBN, it can couple to these optical phonons, giving rise to phonon polaritons that propagate along the material’s surface or within thin flakes. These modes are characterized by extreme spatial confinement and long lifetimes, making them ideal for applications in sub-diffractional imaging, sensing, and on-chip photonic devices.
Research into phonon polaritons in hBN is driven by leading scientific organizations and laboratories worldwide, including the Massachusetts Institute of Technology, Centre National de la Recherche Scientifique, and Max Planck Society. These institutions have contributed to the fundamental understanding of polaritonic behavior in hBN and have demonstrated advanced nanophotonic devices leveraging its unique properties. The ongoing exploration of phonon polaritons in hBN not only deepens our understanding of light-matter interactions at the nanoscale but also paves the way for innovative technologies in IR photonics and quantum information science.
Fundamental Physics of Phonon Polaritons in hBN
Phonon polaritons are hybrid quasiparticles resulting from the strong coupling between photons and optical phonons in polar dielectric materials. In the context of hexagonal boron nitride (hBN), a van der Waals crystal with a layered structure, phonon polaritons exhibit unique properties that have attracted significant attention in nanophotonics and quantum optics. The fundamental physics underlying phonon polaritons in hBN is rooted in the material’s anisotropic crystal structure and its pronounced optical phonon resonances in the mid-infrared (mid-IR) spectral range.
hBN is composed of alternating boron and nitrogen atoms arranged in a hexagonal lattice, leading to strong in-plane covalent bonds and weak interlayer van der Waals interactions. This anisotropy gives rise to two distinct Reststrahlen bands—frequency ranges where the real part of the dielectric permittivity is negative—corresponding to in-plane (Type I) and out-of-plane (Type II) optical phonon modes. Within these bands, hBN supports hyperbolic phonon polaritons, characterized by hyperbolic dispersion relations where the isofrequency surfaces in momentum space are open hyperboloids rather than closed spheres or ellipsoids. This property enables sub-diffractional confinement and propagation of electromagnetic energy, far below the free-space wavelength, making hBN a natural hyperbolic material.
The coupling mechanism between photons and optical phonons in hBN is governed by the material’s polar nature, which allows for strong interaction with the electromagnetic field. When incident light with a frequency within the Reststrahlen bands interacts with hBN, it excites collective oscillations of the lattice ions (optical phonons), which in turn couple to the electromagnetic field, forming phonon polaritons. These quasiparticles can propagate along the hBN surface or within thin flakes, exhibiting long lifetimes and low losses compared to plasmon polaritons in metals, due to the lower intrinsic damping of optical phonons.
The study of phonon polaritons in hBN has been advanced by near-field optical microscopy techniques, which allow direct imaging and manipulation of these modes at the nanoscale. The unique combination of hyperbolic dispersion, strong field confinement, and low loss makes hBN an ideal platform for mid-IR nanophotonics, with potential applications in subwavelength imaging, sensing, and quantum information science. Research in this area is supported by leading scientific organizations such as the Nature Publishing Group and American Physical Society, which regularly publish foundational studies and reviews on the topic.
Crystal Structure and Optical Properties of hBN
Hexagonal boron nitride (hBN) is a layered van der Waals material with a crystal structure analogous to graphite, where boron and nitrogen atoms are arranged in a two-dimensional honeycomb lattice. Each layer consists of alternating boron and nitrogen atoms, strongly bonded covalently within the plane, while adjacent layers are held together by weak van der Waals forces. This anisotropic structure imparts hBN with unique physical and optical properties, making it a prominent platform for studying phonon polaritons.
The optical properties of hBN are dominated by its wide bandgap (approximately 5.9 eV), which renders it transparent in the visible and much of the ultraviolet spectrum. However, in the mid-infrared (mid-IR) spectral range, hBN exhibits strong optical phonon resonances due to the vibrations of the boron and nitrogen atoms. These resonances give rise to two distinct Reststrahlen bands—frequency regions where the real part of the dielectric permittivity is negative—corresponding to in-plane (∼1370–1610 cm−1) and out-of-plane (∼760–825 cm−1) optical phonon modes. Within these bands, hBN supports hyperbolic phonon polaritons (HPhPs), which are hybrid quasiparticles resulting from the strong coupling between photons and optical phonons.
The hyperbolic nature of hBN arises from its anisotropic dielectric tensor: the in-plane and out-of-plane components of the permittivity have opposite signs within the Reststrahlen bands. This leads to hyperbolic dispersion, enabling the propagation of deeply subwavelength polaritonic modes with high confinement and low losses. These properties are fundamentally different from conventional plasmonic materials, where losses are typically higher and confinement is less pronounced. The unique phonon polariton modes in hBN can be directly visualized using advanced near-field optical microscopy techniques, revealing their ability to guide and manipulate light at the nanoscale.
hBN’s exceptional thermal and chemical stability, combined with its atomically smooth surfaces, further enhance its suitability for nanophotonic and quantum optical applications. Its role as a natural hyperbolic material has positioned it at the forefront of research in nanophotonics, enabling the development of novel devices for sub-diffractional imaging, sensing, and on-chip infrared photonics. The study and engineering of phonon polaritons in hBN are actively pursued by leading research institutions and organizations such as the Max Planck Society and the French National Centre for Scientific Research (CNRS), which have contributed significantly to the understanding and application of these unique optical phenomena.
Hyperbolic Dispersion and Anisotropy in hBN
Hexagonal boron nitride (hBN) is a van der Waals material that has garnered significant attention for its unique optical properties, particularly in the context of phonon polaritons—quasiparticles resulting from the strong coupling of photons with optical phonons. A defining feature of hBN is its pronounced optical anisotropy, which arises from its layered crystal structure. This anisotropy leads to hyperbolic dispersion in specific mid-infrared frequency ranges, fundamentally shaping the behavior of phonon polaritons within the material.
In hBN, the dielectric permittivity tensor exhibits opposite signs along the in-plane (parallel to the layers) and out-of-plane (perpendicular to the layers) directions within two distinct spectral regions known as the Reststrahlen bands. In these bands, the real part of the permittivity is negative in one direction and positive in the other, resulting in a hyperbolic dispersion relation. This means that, unlike conventional materials where the isofrequency surfaces are spherical or ellipsoidal, hBN supports open, hyperboloid-shaped isofrequency surfaces. Such hyperbolic materials enable the propagation of highly confined, sub-diffractional polaritonic modes with large wavevectors, which are not accessible in isotropic media.
The hyperbolic nature of hBN is classified as either Type I or Type II, depending on the sign of the permittivity components. In the lower Reststrahlen band (approximately 760–820 cm−1), hBN exhibits Type I hyperbolicity (negative out-of-plane, positive in-plane permittivity), while in the upper Reststrahlen band (approximately 1370–1610 cm−1), it displays Type II hyperbolicity (positive out-of-plane, negative in-plane permittivity). This dual-band hyperbolic behavior is rare among natural materials and is a key reason for hBN’s prominence in nanophotonics and polaritonic research.
The strong anisotropy and hyperbolic dispersion in hBN enable a range of novel optical phenomena, including deeply subwavelength light confinement, negative refraction, and directional propagation of phonon polaritons. These properties are being actively explored for applications in nanophotonic devices, super-resolution imaging, and mid-infrared photonics. Research into hBN’s hyperbolic phonon polaritons is supported by leading scientific organizations and laboratories worldwide, including the Max Planck Society and the French National Centre for Scientific Research (CNRS), both of which have contributed to foundational studies in this field.
Experimental Techniques for Probing Phonon Polaritons
Experimental investigation of phonon polaritons in hexagonal boron nitride (hBN) has advanced rapidly due to the development of sophisticated optical and scanning probe techniques. These methods enable direct visualization and quantitative analysis of polaritonic modes, their dispersion, and their interaction with nanostructures. Among the most prominent techniques are scattering-type scanning near-field optical microscopy (s-SNOM), Fourier-transform infrared (FTIR) spectroscopy, and electron energy loss spectroscopy (EELS).
Scattering-type scanning near-field optical microscopy (s-SNOM) is a powerful tool for probing phonon polaritons at the nanoscale. In s-SNOM, a sharp metallic tip is illuminated with infrared light, generating a highly localized electromagnetic field at the tip apex. When the tip is brought into close proximity with the hBN surface, it launches and detects phonon polaritons, allowing for mapping of their propagation with spatial resolution far below the diffraction limit. This technique has been instrumental in visualizing the hyperbolic polariton modes in hBN, revealing their unique propagation characteristics and subwavelength confinement. The ability of s-SNOM to provide both amplitude and phase information makes it invaluable for studying polariton interference, reflection, and refraction phenomena in hBN nanostructures.
Fourier-transform infrared (FTIR) spectroscopy is another widely used technique for characterizing phonon polaritons in hBN. FTIR measures the absorption and reflection spectra of hBN samples, providing information about the resonant frequencies associated with phonon polariton modes. By analyzing the spectral features, researchers can extract the dielectric function of hBN and identify the frequency ranges where hyperbolic dispersion occurs. FTIR is particularly useful for studying the bulk optical properties and for verifying the presence of Reststrahlen bands, which are frequency regions where hBN supports strong phonon polariton resonances.
Electron energy loss spectroscopy (EELS), often performed in a transmission electron microscope, offers complementary insights by probing the energy loss of electrons transmitted through thin hBN flakes. EELS can resolve the spectral signatures of phonon polaritons with nanometer-scale spatial resolution, enabling the study of polariton modes in confined geometries and at interfaces. This technique is especially valuable for correlating structural and optical properties at the atomic scale.
These experimental approaches, often used in combination, have been crucial in establishing hBN as a model system for phonon polariton research. They have enabled the direct observation of polariton propagation, confinement, and interaction with other materials, paving the way for novel nanophotonic devices based on hBN. Research in this area is supported by leading scientific organizations such as the Nature Publishing Group and American Physical Society, which regularly publish advances in the field.
Theoretical Models and Computational Approaches
The study of phonon polaritons in hexagonal boron nitride (hBN) has advanced significantly through the development of robust theoretical models and computational approaches. Phonon polaritons are hybrid quasiparticles resulting from the strong coupling between optical phonons and electromagnetic fields in polar dielectrics. In hBN, a van der Waals layered material with pronounced anisotropy, these excitations exhibit unique properties such as hyperbolic dispersion, enabling sub-diffractional light confinement and propagation.
The foundational theoretical framework for describing phonon polaritons in hBN is based on Maxwell’s equations coupled with the material’s frequency-dependent dielectric tensor. The dielectric response of hBN is highly anisotropic, with distinct in-plane and out-of-plane permittivities. This anisotropy leads to the emergence of hyperbolic phonon polaritons, where the isofrequency surfaces in momentum space are open hyperboloids rather than closed spheres or ellipsoids. Analytical models often employ the Lorentz oscillator model to represent the phonon contribution to the dielectric function, capturing the resonant behavior near the transverse and longitudinal optical phonon frequencies.
To accurately predict and analyze phonon polariton behavior in realistic hBN structures, computational electromagnetic methods are widely used. Finite-difference time-domain (FDTD) and finite element method (FEM) simulations allow for the modeling of complex geometries, including nanostructured hBN and heterostructures with other two-dimensional materials. These numerical approaches can incorporate the full anisotropic dielectric tensor and account for boundary conditions, enabling the study of polariton propagation, confinement, and interaction with nanostructures.
First-principles calculations, such as density functional theory (DFT) and density functional perturbation theory (DFPT), are employed to obtain accurate phonon dispersion relations and dielectric properties of hBN. These ab initio methods provide essential input parameters for both analytical and numerical models, ensuring that the theoretical predictions align with experimental observations. The combination of DFT-derived material parameters with electromagnetic simulations has proven crucial for understanding the impact of defects, layer thickness, and isotopic composition on phonon polariton characteristics.
Furthermore, the development of nonlocal and quantum models is an emerging area, particularly relevant for describing phonon polaritons in ultra-thin hBN layers and at the nanoscale. These models go beyond the local dielectric response, incorporating spatial dispersion and quantum confinement effects, which become significant as device dimensions approach the nanometer regime.
The synergy between analytical theory, computational electromagnetics, and first-principles calculations has established a comprehensive toolkit for exploring and engineering phonon polaritons in hBN. This multidisciplinary approach is supported by leading research institutions and organizations such as the Max Planck Society and the French National Centre for Scientific Research (CNRS), which have contributed extensively to both theoretical and experimental advancements in this field.
Applications in Nanoscale Photonics and Sensing
Phonon polaritons in hexagonal boron nitride (hBN) have emerged as a transformative platform for applications in nanoscale photonics and sensing, owing to their unique ability to confine and guide electromagnetic energy at deeply subwavelength scales. These hybrid quasiparticles arise from the strong coupling between infrared photons and optical phonons in polar dielectric materials such as hBN, which is a van der Waals crystal characterized by its wide bandgap, high thermal stability, and pronounced optical anisotropy.
One of the most significant advantages of hBN is its support for hyperbolic phonon polaritons (HPPs) within two distinct mid-infrared spectral bands, known as the Reststrahlen bands. In these frequency ranges, hBN exhibits opposite signs of permittivity along its in-plane and out-of-plane axes, resulting in hyperbolic dispersion. This property enables the propagation of highly confined polaritonic modes with wavelengths much shorter than that of free-space light, facilitating the design of nanophotonic devices with unprecedented spatial resolution and field enhancement capabilities.
In nanoscale photonics, hBN-based structures have been utilized to realize waveguides, resonators, and nanoantennas that operate in the mid-infrared regime. These devices leverage the low-loss propagation and strong field confinement of HPPs, making them ideal for on-chip optical circuits and compact photonic components. The ability to pattern hBN into nanostructures further allows for the engineering of polaritonic dispersion and the tailoring of light-matter interactions at the nanoscale. Such advances are critical for the development of next-generation infrared photonic technologies, including compact spectrometers and tunable filters.
In the realm of sensing, the extreme field confinement and enhanced local density of optical states associated with hBN phonon polaritons enable highly sensitive detection of molecular vibrations and chemical species. When molecules are placed in close proximity to hBN nanostructures, their vibrational fingerprints can be strongly amplified via surface-enhanced infrared absorption (SEIRA) or tip-enhanced near-field spectroscopy. This capability is particularly valuable for label-free biosensing, environmental monitoring, and the identification of trace analytes at the nanoscale.
The integration of hBN with other two-dimensional materials, such as graphene, further expands the functional landscape of polaritonic devices, allowing for active modulation and tunability of polariton properties. As research continues, the unique phonon polariton phenomena in hBN are expected to underpin a new generation of miniaturized, high-performance photonic and sensing platforms, with broad implications for quantum optics, optoelectronics, and materials science. For foundational research and material standards, National Institute of Standards and Technology (NIST) and American Physical Society (APS) are among the leading organizations supporting advances in this field.
Integration with Other 2D Materials and Heterostructures
The integration of hexagonal boron nitride (hBN) with other two-dimensional (2D) materials has emerged as a powerful strategy to engineer and exploit phonon polaritons for advanced nanophotonic applications. hBN is renowned for its ability to support low-loss, highly confined phonon polaritons in the mid-infrared spectral range, owing to its natural hyperbolic dispersion and strong anisotropy. When combined with other 2D materials—such as graphene, transition metal dichalcogenides (TMDs), or black phosphorus—hBN serves as both an active and passive component in van der Waals heterostructures, enabling new functionalities and tunability in polaritonic devices.
One of the most prominent examples is the integration of hBN with graphene. Graphene, a single layer of carbon atoms with exceptional electronic and optical properties, can be stacked with hBN to form heterostructures where the phonon polaritons in hBN interact with the plasmon polaritons in graphene. This interaction allows for active electrical tuning of the polaritonic response, as the carrier density in graphene can be modulated via gating, thereby influencing the propagation and confinement of phonon polaritons in the adjacent hBN layer. Such hybrid systems have demonstrated tunable mid-infrared nano-optics, paving the way for reconfigurable photonic circuits and sensors.
Beyond graphene, hBN has been integrated with TMDs (such as MoS2 and WS2) and black phosphorus to create heterostructures with tailored optical responses. These combinations leverage the unique excitonic and anisotropic properties of the constituent materials, enabling strong light-matter interactions and the possibility of coupling between excitons and phonon polaritons. The atomically flat and chemically inert nature of hBN also makes it an ideal substrate and encapsulation layer, preserving the intrinsic properties of sensitive 2D materials and enhancing device stability.
The fabrication of such heterostructures typically relies on mechanical exfoliation and deterministic transfer techniques, allowing precise control over layer thickness and stacking order. This versatility has led to the demonstration of novel phenomena, including negative refraction, sub-diffractional imaging, and ultra-confined waveguiding, all mediated by phonon polaritons in hBN-based heterostructures.
The ongoing research in this field is supported by major scientific organizations and collaborative initiatives, such as the Centre National de la Recherche Scientifique (CNRS) and the Max Planck Society, which have contributed significantly to the understanding and development of 2D material heterostructures. As integration techniques and material quality continue to improve, the synergy between hBN and other 2D materials is expected to unlock new paradigms in nanophotonics and quantum technologies.
Challenges and Limitations in Current Research
Research into phonon polaritons in hexagonal boron nitride (hBN) has advanced rapidly, yet several challenges and limitations persist, impeding both fundamental understanding and practical applications. One of the primary obstacles is the fabrication of high-quality, large-area hBN crystals. The synthesis of hBN with minimal defects and uniform thickness is crucial, as imperfections can significantly affect phonon polariton propagation and confinement. Current methods, such as chemical vapor deposition (CVD) and mechanical exfoliation, often yield samples with variable quality and limited scalability, restricting reproducibility and device integration.
Another significant challenge lies in the precise control and characterization of phonon polaritons at the nanoscale. Techniques like scattering-type scanning near-field optical microscopy (s-SNOM) have enabled visualization of polaritonic modes, but these methods require sophisticated instrumentation and expertise, limiting widespread adoption. Furthermore, the interpretation of near-field data can be complicated by tip-sample interactions and substrate effects, introducing uncertainties in the extracted material parameters.
Theoretical modeling of phonon polaritons in hBN also faces limitations. While continuum electrodynamics and first-principles calculations provide valuable insights, they often rely on idealized assumptions that may not fully capture the complexities of real materials, such as anisotropy, edge effects, and environmental influences. Bridging the gap between theory and experiment remains a persistent issue, particularly when exploring novel heterostructures or non-trivial geometries.
Integration of hBN-based polaritonic devices with existing photonic and electronic platforms presents additional hurdles. The strong confinement and hyperbolic dispersion of phonon polaritons in hBN are promising for sub-diffractional optics, but efficient coupling to external circuits and waveguides is non-trivial. Loss mechanisms, including phonon scattering and substrate-induced damping, further limit device performance and operational bandwidth.
Finally, the field faces a lack of standardized protocols for material characterization and device benchmarking. This hampers direct comparison between studies and slows the translation of laboratory findings into technological innovations. Addressing these challenges will require coordinated efforts across materials science, nanofabrication, and optical engineering communities, as well as support from major research organizations such as the Nature Publishing Group and American Physical Society, which play pivotal roles in disseminating advances and fostering collaboration.
Future Directions and Emerging Opportunities
The field of phonon polaritons in hexagonal boron nitride (hBN) is rapidly evolving, with future directions and emerging opportunities poised to significantly impact nanophotonics, quantum technologies, and materials science. hBN’s unique ability to support hyperbolic phonon polaritons—hybrid quasiparticles arising from strong coupling between infrared photons and optical phonons—enables sub-diffractional light confinement and propagation at mid-infrared to terahertz frequencies. This property is driving research into next-generation photonic devices and novel quantum platforms.
One promising direction is the integration of hBN-based phonon polariton structures with other two-dimensional (2D) materials, such as graphene and transition metal dichalcogenides. Such heterostructures can combine the low-loss, highly confined polaritonic modes of hBN with the tunable electronic and optical properties of adjacent layers, enabling active modulation and new device functionalities. This approach is being explored by leading research institutions and collaborative initiatives, including those supported by Nature Publishing Group and Centre National de la Recherche Scientifique (CNRS).
Another emerging opportunity lies in the development of quantum nanophotonics platforms. hBN’s hyperbolic phonon polaritons can enhance light-matter interactions at the nanoscale, which is crucial for single-photon sources, quantum sensors, and strong-coupling experiments. The material’s compatibility with quantum emitters, such as color centers in hBN itself, opens pathways for integrated quantum photonic circuits. Research in this area is being advanced by organizations like Max Planck Society and Massachusetts Institute of Technology (MIT).
On the technological front, scalable fabrication and patterning of hBN nanostructures remain key challenges and opportunities. Advances in nanolithography, chemical vapor deposition, and transfer techniques are expected to enable large-area, high-quality hBN films and devices. This will facilitate the commercialization of hBN-based polaritonic components for applications in sensing, imaging, and on-chip optical communication. Standardization and material quality control are being addressed by international bodies such as the International Organization for Standardization (ISO).
Finally, the exploration of new physical phenomena—such as topological polaritons, nonlocal effects, and strong coupling regimes—will continue to expand the fundamental understanding and application space of phonon polaritons in hBN. As research progresses, interdisciplinary collaboration among physicists, materials scientists, and engineers will be essential to fully realize the transformative potential of this platform.
Sources & References
- Massachusetts Institute of Technology
- Centre National de la Recherche Scientifique
- Max Planck Society
- Nature Publishing Group
- Max Planck Society
- National Institute of Standards and Technology (NIST)
- International Organization for Standardization (ISO)